Rate of cerebral blood flow (CBF). CBF rates are largely determined by the cerebral
metabolic rate for oxygen consumption; there is
exquisite coupling of blood flow and metabolism on a
regional basis. The global blood flow to the brain
remains fairly stable for a given physiologic state
(e.g., awake adult). Anesthetics and hypothermia
tend to decrease metabolism throughout the brain and
thereby reduce global CBF.
The global CBF in adults is approximately 50 mL/100g/minute. This global measure is composed of flow
from two very different regions: the gray
matter, which is where neuronal cell bodies and
synapses are located and has a blood flow of 75 mL/100
g/minute, and the white matter, which consists
mainly of fiber tracts and has a blood flow of 20 mL/100
g/minute. The higher blood flow to gray matter is
primarily due to its greater metabolic rate.
The global CBF of children is approximately 95 mL/100
g/minute, which is higher than that of adults. In
contrast, infants have a slightly lower CBF than
adults (40 mL/100 g/minute).
Spinal cord blood flow has been less extensively
studied; the gray matter has a rate of 60 mL/100
g/minute and the white matter a rate of 20 mL/100
g/minute.
Regulation of CBF.
Regional flow and metabolism coupling depends on the
buildup of metabolites that cause local dilatation
of the microvessels.
The precise mechanism of this coupling is unknown.
It may be due to the buildup of K or H in the
extracellular fluid surrounding the arterioles.
Other agents that may mediate flow and metabolism
coupling include nitric oxide (NO), calcium,
adenosine, and eicosanoids such as thromboxane and
prostaglandin. A combination of these factors likely
contributes to coupling.
NO is a vasodilator that is released locally by the
vascular endothelial cells. This vasodilatation is
important for vascular regulation throughout the
body, but the precise role of NO in the control of
CBF remains to be determined. It is likely to be an
important regulator of local CBF, perhaps by
affecting arterioles upstream of the microvessels
dilated by metabolic factors.
Carbon dioxide (CO2) enhances vasodilatation and
increases CBF. CO2 is hydrated with the help of
carbonic anhydrase leading to an acidification. This
reduction in pH is thought to cause the
vasodilatation. When CO2 is halved from 40 to 20 mm
Hg, the CBF is reduced by approximately half (Figure-1).
Hyperventilation leads to a reduction in CO2, an
increase in pH, and thereby a reduction in CBF. If
hyperventilation is maintained over a period of 6 to
8 hours, the pH returns to normal due to bicarbonate
transport and CBF returns to its prehyperventilation
levels. Thus, hyperventilation is useful for only
short periods of time.
(1) If hyperventilation is discontinued abruptly,
the increase in CO2 to the normal level, in the
presence of reduced HCO3, leads to acidosis and an
above
normal increase in CBF. This can be a critical
problem when hyperventilation is used to reduce
intracranial pressure (ICP).
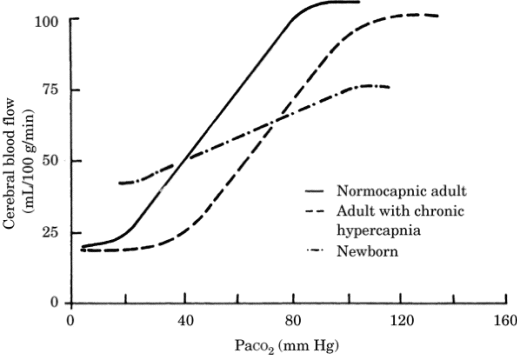
Figure-1. Relationship between cerebral blood flow
and arterial carbon dioxide tension (PaCO2) in the
normocapnic adult, the hypercapnic adult, and the
newborn.
(2) It is possible that extreme hyperventilation to
levels below 20 mm Hg can lead to ischemia due to
vasoconstriction; thus, hyperventilation below 30 mm
Hg is not recommended clinically.
Autoregulation allows CBF to remain constant if the
cerebral perfusion pressure (CPP) varies between 50
and 150 mm Hg. Signs of cerebral ischemia are seen
below 50 mm Hg; above 150 mm Hg, disruption of the
blood-brain barrier (BBB) and cerebral edema may
occur. The adjustment of flow to abrupt changes in
pressure requires 30 to 180 seconds (Figure-2).
The mechanism of autoregulation is not completely
understood but is likely to be a combination of
effects including myogenic and metabolic factors.
(1) Myogenic activity of the vessel wall musculature
occurs in response to increased distending pressure.
In isolated vessels, when the vessel wall is
stretched, as it would be by increased blood
pressure, the smooth muscle contracts, causing a
vasoconstriction that reduces flow. This balances
out the increase in blood flow due to the increased
pressure, resulting in little net change in blood
flow.
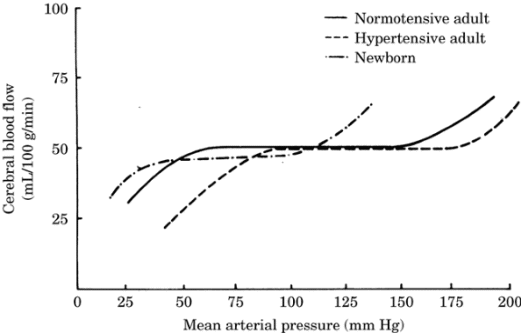
Figure-2. Autoregulatory curve of the cerebral
vasculature in the normotensive adult, the
hypertensive adult, and the newborn.
(2) The metabolic theory states that reduced
pressure leads to reduced flow and the buildup of
metabolites. This buildup in metabolites and the
decrease in local pH lead to a local vasodilatation
and thereby an increase in blood flow back to
normal.
(3) Autoregulation can be impaired by hypoxia,
ischemia, hypercapnia, trauma, and certain
anesthetic agents.
(4) Patients who are chronically hypertensive or
have a high sympathetic tone have a shift in the
autoregulatory curve to the right. They may
demonstrate signs of ischemia due to reduced blood
flow at pressures above the lower limit for
normotensive individuals.
The partial pressure of oxygen (PaO2) has little
effect on global CBF until it falls below 50 mm Hg.
At this point, a dramatic increase in blood flow
with further reductions in PaO2 occurs. Since the
oxygen-carrying capacity of blood is high, a
critical reduction in the oxygen content of the
blood might not occur until the Pao2 falls below a
threshold of 50 mm Hg (Figure-3).
Neurogenic factors including adrenergic,
cholinergic, and serotonergic systems also influence
CBF. Their greatest influence is on larger blood
vessels.
The hematocrit alters blood viscosity and thereby
can affect blood flow. A low hematocrit can increase
blood flow by decreasing blood viscosity.
Hypothermia decreases neuronal metabolism and
thereby reduces CBF; hyperthermia has the opposite
effect.
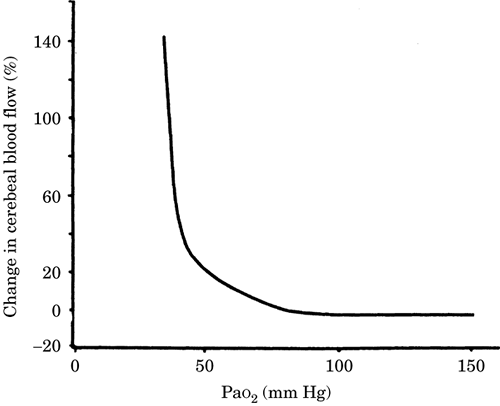
Figure-3. Relationship between cerebral blood flow
and PaO2, arterial oxygen tension.
The metabolic rate of neurons surrounding the
microvasculature regulates regional blood flow. If
the activity of these neurons increases, their
metabolic rate increases, and the blood flow to that
region increases to meet the demand for oxygen and
glucose. This property has been exploited using
positron emission tomography to functionally map
brain activity.
|