
Patients with neurologic disease
undergoing surgical procedures have increased risk
of ischemic/hypoxic damage to the central nervous
system (CNS). This risk may be related to
hemodynamic/embolic events associated with a
non-neurosurgical operation (e.g., patients with
significant carotid stenosis undergoing
cardiopulmonary bypass [CPB] procedures) or the risk
may be inherent in the neurosurgical procedure
itself (e.g., temporary clipping of feeding artery
during cerebral aneurysm surgery). Intraoperative
neurophysiologic monitoring may improve patient
outcome by (a) allowing early diagnosis of
ischemia/hypoxia before irreversible damage occurs
and (b) enabling surgeons to provide optimal
operative treatment as indicated by the monitoring
parameter.
Although not universally adopted, neurophysiologic
monitoring has become routine for some surgical
procedures in many centers.
Broadly speaking, the brain can be monitored in
terms of (a) function, (b) blood flow, and (c)
metabolism (Tables-1, 2 and 3).
I. Monitoring of
function
Electroencephalography.
Summation of the excitatory postsynaptic potential
generated by the pyramidal cells of the cerebral
cortex gives rise to the electrical activity of the
brain, which can be recorded as an
electroencephalogram (EEG). The EEG comprises many
underlying components with different frequencies and
harmonics. The component waves are typically
classified according to the respective frequencies
(Table-4). This electrical activity is volume
conducted and can be recorded from the scalp and
forehead, using surface or needle electrodes.
Recording techniques.
Because the scalp has no electrically neutral area,
EEG is typically recorded using a montage (electrode
arrangement) with bipolar recording. Thus, both
electrodes are active, and the polarity of the
signal recorded depends on the arbitrary designation
of recording versus referential electrode. Other
electrical activities generated in the body such as
electromyographic and electrocardiographic
potentials are minimized but not eliminated using
common-mode rejection which rejects the electrical
signals that are measured in both recording sites
(common) in comparison to a third ground electrode.
The number of channels used and the placement of
electrodes determine the specificity of the EEG as a
monitor of the occurrence of regional ischemia. The
gold standard for raw EEG recording is 16-channel
recording (eight channels for each hemisphere) with
electrodes placed according to the International
10-20 System (Figure-1). With the exception of
monitoring in carotid endarterectomy (CEA) in some
centers, 16-channel EEG recording is seldom
performed intraoperatively because of its relative
complexity and the inaccessibility of recording
sites in intracranial surgical procedures.
Table-1. Monitoring
of function |
Electroencephalogram |
Raw
electroencephalogram |
Computerized
processed |
Compressed
spectral array |
Density
spectral array |
Aperiodic
analysis |
Bispectral Analysis |
Evoked
potentials |
Sensory evoked
potentials: |
Somatosensory EP |
Brain stem
auditory EP |
Visual EP |
Motor evoked
potentials |
Transcranial
magnetic MEP |
Transcranial
electric MEP |
Direct
spinal cord stimulation |
Electromyography |
Cranial nerve
functions (V, VII, IX, X, XI, XII) |
EP: evoked
potential; MEP: motor evoked potential. |
To simplify recording and
interpretation of EEG, most EEG machines designed
for intraoperative monitoring use two- to
four-channel recording with computer processing to
simplify the output. Although different vendors use
different algorithms, the basic premise is to filter
out the high frequency activity (likely to be
artifacts or interference), typically at 30 Hz. The
raw EEG is then separated into its component waves
using Fast Fourier Transform and then they are
grouped together according to the frequency
spectrum. Thus, raw EEG recorded in a time domain is
displayed in a frequency domain. The resultant power
(square of the amplitude of the EEG wave) spectrum
can be displayed in a number of ways, the most
common ones being compressed spectral array or
density spectral array with either the peaks and
valleys or the density of the gray scale
representing the power of the spectrum. Aperiodic
analysis is another method of EEG processing that
tracks each wave and plots it as a telephone pole
with its height representing the amplitude or power
of the wave.
Table -2. Monitoring
of flow/pressure |
Cerebral blood
flow |
Nitrous oxide
wash-in |
Radioactive xenon
clearance |
Laser Doppler blood
flow |
Transcranial
Doppler |
Intracranial
pressure |
Intraventricular
catheter |
Fiberoptic
intraparenchymal catheter |
Subarachnoid bolt |
Epidural catheter |
Table-3. Monitoring
of metabolism |
Invasive monitor |
Intracerebral Po2
electrode (Paratrend, Licox) |
Noninvasive
monitor |
Transcranial
cerebral oximetry (near-infrared
spectroscopy) |
Jugular venous
oximetry |
Interpretation of EEG
The EEG is a random activity reflecting the state of
arousal and metabolic activity. The generation of
electrical activity is an energy-requiring process
that depends on an adequate supply of substrates
including oxygen and glucose. Thus, significant
reductions of blood flow, oxygen, or glucose all
lead to depression of EEG activity. Gradual
reduction of cerebral blood flow (CBF) can be
correlated with characteristic changes in EEG and
constitutes the most frequent underlying indication
for EEG monitoring.
Table-4.
Electroencephalogram |
Beta |
13-30 Hz |
High frequency, low
amplitude, dominant during awake state |
Alpha |
9-12 Hz |
Medium frequency,
higher amplitude seen in occipital cortex
with eyes closed while awake |
Theta |
4-8 Hz |
Low frequency, not
predominant in any condition |
Delta |
0-4 Hz |
Very low frequency,
low to high amplitude signifies depressed
functions, consistent with deep coma (cause
can be anesthesia, metabolic, or hypoxia) |
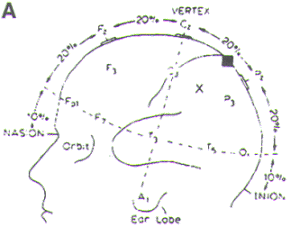 |
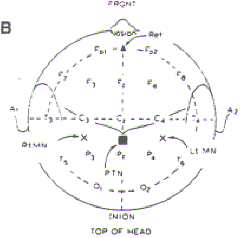 |
Figure-1. The International 10-20 System for
placement of EEG electrodes. It is based on dividing
each head circumference (anteroposterior from nasion
to inion over the vertex, coronal from tragus of one
ear to the other) into two halves and then
subdividing each half (50%) into 10%, 20%, 20%
sectors. Fp, frontal pole; F, frontal; C, central;
P, parietal; T, temporal; A, ear lobe; M, mastoid
(not shown). Odd number subscripts denote the left
hemisphere, and even numbers denote the right
hemisphere. |
Awake EEG is dominated by beta activity with
high-frequency and low-amplitude waves. With the
onset of ischemia/hypoxia, initially a transient
increase in beta activity occurs, followed by
development of slow waves (theta and delta) with
large amplitude, disappearance of beta activity, and
eventually the occurrence of delta waves with low
amplitude. The ischemic changes can progress to
suppression of electrical activity with an
occasional burst of activity
(burst suppression) and finally to complete
electrical silence with flat EEG, signaling the
onset of irreversible damage. Thus, the sudden
development of delta waves coincident with a
surgical maneuver such as cross-clamping of the
common carotid artery would warn the
anesthesiologist and the surgeon that the patient is
at risk of cerebral injury. In the region of the
ischemic penumbra where blood flow is inadequate to
generate electrical activity but sufficient to
maintain neuronal viability for a period of time,
EEG has poor predictive power for brain damage.
Thus, EEG is sensitive to the occurrence of ischemia
but lacks specificity as a diagnostic test for
irreversible damage. In general, the quicker the
onset of the ischemic EEG changes, the higher the
probability for irreversible damage.
Confounding factors. The observed changes in EEG
with ischemia/hypoxia are not unique, and similar
changes occur with anesthetic-induced metabolic
depression, albeit in a reversible manner. Most
intravenous (with the exception of ketamine) and
inhalation anesthetics cause a dose-dependent
depression of EEG and virtually all of them can
produce a burst-suppression pattern on EEG.
Similarly, hypothermia decreases cerebral metabolism
and causes slowing of the EEG. EEG changes,
therefore, should always be interpreted in concert
with other physiologic variables, not in isolation.
Indications for EEG monitoring
All surgical procedures that potentially place the
brain at risk are theoretically amenable to EEG
monitoring. In practice, EEG monitoring is often
difficult to perform during intracranial procedures
because of the lack of access to scalp recording.
The use of needle electrodes may ameliorate this
problem. CEA, which places the ipsilateral
hemisphere at risk during cross-clamping of the
common carotid artery, is the most frequent
indication for EEG monitoring. When EEG changes
indicate that CBF is inadequate, the placement of an
intraluminal shunt restores blood flow.
Some anesthesiologists and surgeons utilize
anesthesia-induced metabolic suppression to provide
cerebral protection during risky procedures. EEG
monitoring in these circumstances allows optimal
metabolic suppression with anesthesia administered
by titration to achieve burst suppression. The main
indications for EEG monitoring are listed in Table-5.
Table -5. Indications for electroencephalogram
monitoring |
Carotid endarterectomy |
Cerebral aneurysm surgery when temporary clipping is
used |
Cardiopulmonary bypass procedures |
Extracranial-intracranial bypass procedures
|
Deliberate metabolic suppression for cerebral
protection |
Although uncommon in the anesthetized patient,
occult seizures occur frequently in patients with
head injury in the intensive care unit (ICU). These
seizures, which may worsen the outcome, may be
detectable only with continuous EEG monitoring.
Although treatment of these seizures is likely
beneficial, it is yet to be determined whether
continuous EEG monitoring in the ICU will improve
outcome in a cost-effective manner.
Bispectral analysis. The bispectral index is a
derived EEG parameter designed not to detect
ischemia but to monitor the degree of hypnosis.
Based on both power spectrum analysis and phase
change or coherence of the different frequencies,
the value is normalized to a range of 0 to 100. A
value between 40 and 60 is considered adequate
hypnosis to prevent possible recall, whereas a value
above 80 is consistent with impending emergence from
anesthesia. Strictly speaking, this is not a
parameter used to preserve the integrity of the CNS
but is derived from the raw EEG and, as such, it is
potentially influenced by the occurrence of ischemia
that results in changes in the EEG. The frontal
location of electrode placement, however, renders
the bispectral index less sensitive to development
of regional ischemia.
Evoked potential monitoring
Sensory evoked potentials (SEPs). SEP is a
time-locked, event-related, pathway-specific
electroencephalographic activity generated in
response to a specific stimulus such as electrical
stimuli applied to the median nerve. The typical
peaks and troughs are described by their polarity
and latency (Figure-2). For example, the cortical
negative peak that typically occurs 20 msec after
stimulation of the median nerve is called N20.
Alternatively, it is numbered according to the
sequence in which it is generated; thus, the first
positive wave that occurs following posterior tibial
nerve stimulation is called P1. The amplitude of
evoked potential waves is small relative to
conventional
EEG and is not easily visualized without computer
averaging of repetitive stimuli. SEPs are
anatomically pathway specific and theoretically
assess only the integrity of the pathway monitored.
The contrast between conventional EEG and SEP is
summarized in Table -6.
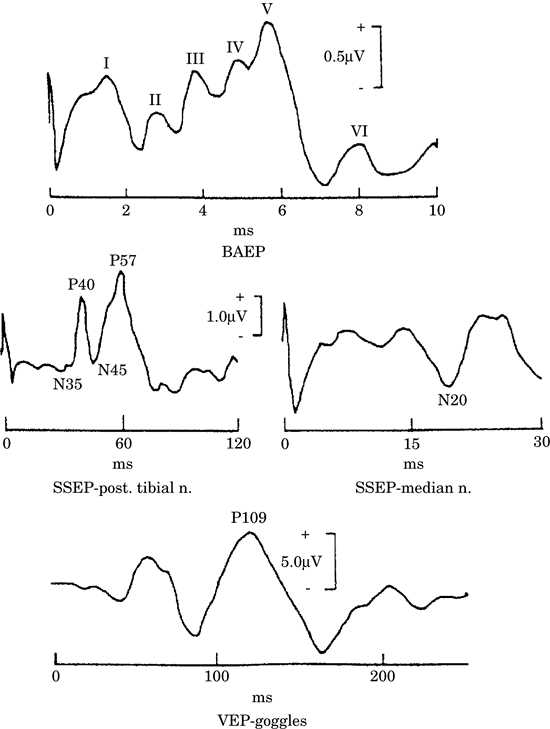 |
Figure -2. Common modalities of sensory evoked
responses. BAEP: brain stem auditory evoked
potential; SSEP-median n, somatosensory evoked
potential with median nerve stimulation; SSEP-post
tibial n, somatosensory evoked potential with
posterior tibial nerve stimulation; VEP-goggles,
visual evoked potential in response to
stimulation with light-emitted diode
goggles. |
SEP can be recorded in response to stimulation of
any sensory nerve, cranial or peripheral. The common
modalities of evoked potentials
used in clinical practice are (a) somatosensory
evoked potentials (SSEPs), (b) visual evoked
potentials (VEPs), and (c) brain stem auditory
evoked potentials (BAEPs). Of these three, VEP is
profoundly influenced by inhalation anesthesia, is
difficult to perform, and is seldom utilized as an
intraoperative monitor. The SSEPs recorded in
response to stimulation of the median nerve, ulnar
nerve, and posterior tibial nerve monitor the
integrity of the respective pathways from the
periphery to the cortex. They are a routine monitor
for surgical procedures on the spinal column with
potential risk to the spinal cord such as scoliosis
surgery.
Table -6. Difference between sensory evoked
potential (SEP) and electroencephalogram (EEG) |
SEP |
EEG |
Event-related |
Random |
Anatomically
pathway specific |
Primarily cortical
activity |
Monitors integrity
of the pathway |
Monitors only the
cortex |
Small amplitude |
Large amplitude |
Requires computer
averaging |
No averaging required |
Resistant to
intravenous anesthetics, recordable during
inhaled anesthetics |
Abolished by high dose
intravenous or inhalation anesthetics |
SEPs are also used during CEA and cerebral aneurysm
surgery with the theoretical advantage over EEG that
even subcortical ischemia can be detected.
Indications for different SEP-monitoring modalities
are summarized in Table-7.
Table-7. Indications for sensory evoked potential
(SEP) monitoring |
SSEP monitoring |
Spinal column surgery |
Carotid endarterectomy |
Cerebral aneurysm surgery |
BAEP monitoring |
Acoustic schwannoma |
Vertebral-basilar aneurysms |
Other posterior fossa procedures |
SSEP, somatosensory evoked potential; BAEP, brain
stem auditory evoked potential. |
BAEPs include a series of seven short-latency peaks
generated in response to stimulation of the auditory
(VIII) nerve, typically with repetitive clicks
delivered to the ears with a head phone or
ear-inserted transducers. A specific neurogenerator
produces each peak, numbered with a Roman numeral.
Examination of the change in latency interval
between various peaks can localize the specific site
of the injury. Monitoring BAEPs during acoustic
schwannoma surgery, when feasible, has been shown to
help preserve the integrity of the auditory nerve.
Although the neurogenerators are specific to the
VIII nerve, BAEP monitoring has also been used to
reflect the general well-being of the brain stem in
posterior fossa procedures.
Interpretation of evoked potentials. As with EEG,
ischemia/hypoxia leads to depression of conduction
with resultant decrease in amplitude and increase in
latency of the specific peaks. For SSEPs, 50%
reduction in amplitude from baseline in response to
a specific surgical maneuver is generally accepted
to be a significant change warranting alteration of
surgical strategy to avert potential damage. Some
centers also consider a 10% increase in latency of
SSEP signals to be significant. For BAEP, an
increase in latency of more than 1 msec,
particularly in wave V, is considered to be
clinically significant. In addition, a 50% decline
in amplitude is a concern, and a loss of wave V is
strongly predictive of postoperative hearing loss.
Confounding factors. As with EEG, anesthetic agents
influence cortical evoked potentials. Unlike EEG, SSEPs resist the influence of intravenous agents.
Although the amplitude may be slightly reduced and
the latency increased, cortical SSEPs can be
recorded even during deep barbiturate-induced coma
with isoelectric EEG. In contrast, inhalation
anesthetics cause a dose-related decrease in
amplitude and increase in latency. With high doses,
SSEP can become unrecordable. Nitrous oxide also has
a profound depressant effect on the amplitude of
SSEP, particularly when used in combination with an
inhalation anesthetic. Therefore, the combination of
inhalation anesthetic and nitrous oxide is avoided.
Intraoperative recording of SSEP is best
accomplished using an intravenous anesthetic
technique or low-dose inhalation anesthetic (<1
minimum alveolar concentration [MAC]). Opioids have
negligible effects on SSEP. Ketamine and etomidate
have a paradoxical effect of augmenting the
amplitude of SSEP and could make monitoring possible
in patients with otherwise unrecordable SSEP. BAEP,
unlike cortical SSEP, resists the influence of
anesthetic agents
and can be recorded even during high-dose inhalation
anesthetics. For surgical procedures on the spinal
column, evoked potentials can also be recorded
directly from the epidural space; these potentials
are robust and can be recorded regardless of
anesthetic agents used. As with EEG, hypothermia
also decreases amplitude and increases latency.
Motor evoked potentials. Because SSEP monitors only
the integrity of the sensory pathway, it is
theoretically possible to miss an injury
specifically affecting the motor pathway but sparing
the sensory tracts. Thus, motor evoked potential
(MEP) recording was introduced into clinical
practice to complement SSEP recording. The MEP is
basically an electromyographic potential recorded
over muscles in the hand or foot in response to
depolarization of the motor cortex (Figure-3).
Depolarization can be achieved using transcranial
magnetic or electrical stimulation.
Unfortunately, anesthetic agents profoundly
influence both modalities, rendering the
transcranial magnetic stimulation essentially
unrecordable during anesthesia and the electrical
stimulation recordable only during total intravenous
anesthesia. The use of a train-of-four stimuli has
augmented MEP and makes it easier to record under
general anesthesia. In contrast to SSEP, the
relatively large voltage of MEP abrogates the need
for averaging and provides almost instant feedback.
In many centers, MEP monitoring is used in addition
to SSEP during operations on the spinal column. MEPs
cannot be recorded in the presence of neuromuscular
blockade.
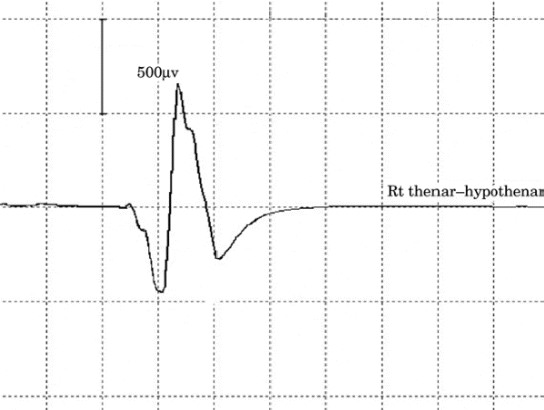 |
Figure-3. Motor evoked potential from stimulation
of left cranium. |
Spontaneous electromyography. Although not
technically an evoked potential, spontaneous
electromyography (EMG) is frequently recorded during
surgical procedures of both the cervical spine and
lumbar spine. Irritation of peripheral nerves, such
as spinal nerve roots, invokes immediate motor
activity in muscles that should otherwise be silent
under general anesthesia. Spontaneous EMG is
particularly useful when the surgeon is working
around nerve roots and needs immediate feedback
regarding injury or irritation to them.
Monitoring cranial nerve functions. Operations in
the posterior fossa and the lower brain stem are
associated with potential injury to the cranial
nerves. Monitoring the electromyographic potential
of the cranial nerves with motor components (V, VII,
IX, X, XI, XII) can preserve the integrity of these
cranial nerves. Two types of potentials can be
recorded: spontaneous and evoked activity. Measuring
spontaneous activity can detect injury potential,
signifying accidental surgical trespass. Evoking the
nerve with electrical stimulation facilitates
identification and thus preservation of the cranial
nerve. Although EMG theoretically can be recorded
during partial neuromuscular blockade, it is best
not to administer any muscle relaxants during
cranial nerve monitoring.
II. Monitoring of CBF and intracranial pressure
(ICP)
Absolute CBF. Most methods of CBF measurement are
not applicable to intraoperative monitoring. Nitrous
oxide wash-in is the classic method of measuring
global hemispheric CBF, but it necessitates cannulation of the jugular bulb and clinically is
cumbersome to use. Radioactive xenon clearance is a
method that allows noninvasive measurement of
regional CBF. Although some centers use it
clinically to determine adequacy of hemispheric CBF
during CEA, it is primarily a research tool.
Relative CBF. Other tools used for real-time
evaluation of relative changes in CBF include laser
Doppler flowmetry (LDF) and transcranial Doppler (TCD)
sonography.
LDF. This tool is a parenchymal or surface Doppler
probe that measures tissue local CBF in a
quantitative manner. However, the flow can be
expressed only in a relative manner in arbitrary
units. Because the volume of tissue monitored is
limited to 1 mm, its clinical use, both in the
operating room (OR) and the ICU, remains limited.
Its insertion also requires a burr hole. Its
incorporation
into the intracranial monitor may increase its
functionality.
TCD sonography. This procedure allows measurement of
CBF velocity in the major vessels of the Circle of
Willis noninvasively and continuously. For
intraoperative purposes, velocity in the middle
cerebral artery (Vmca) is measured transtemporally
over the zygomatic arch. Although it does not derive
the absolute CBF, it can measure relative changes in
CBF in a quantitative manner. The waveform profile
also provides a qualitative assessment of
ICP/cerebral perfusion pressure (CPP). In addition,
the occurrence of air or particulate emboli can be
detected. TCD can be used to determine cerebral
autoregulation and carbon dioxide (CO2) reactivity.
Physiological principles of TCD
(1) The diameter of the basal cerebral arteries is
constant. Flow velocity is proportional to CBF only
when the diameter of the insonated vessel and the
angle of insonation remain constant. There is
considerable evidence to suggest that the basal
cerebral arteries, as conductance vessels, do not
dilate or constrict as the vascular resistance
changes. Angiographic and CO2 reactivity studies
confirm that changes in CO2 tension and blood
pressure have negligible influence on the diameter
of the proximal basal arteries. Intravenous and
inhaled anesthetic agents do not constrict or dilate
the middle cerebral artery (MCA) appreciably.
Probably the only clinically important situation in
which the basal cerebral vessels vasoconstrict is
when vasospasm occurs as a complication of a
subarachnoid hemorrhage. Vasospasm renders the
relationship between CBF velocity and CBF invalid;
as the vessel constricts, the flow velocity
increases but the CBF actually decreases. This
increase in flow velocity with constriction of the
basal cerebral artery represents one of the most
important and established uses of the TCD. Once
angiography confirms the diagnosis of vasospasm, TCD
can track the patient's response to therapy and the
time course of resolution of the vasospasm.
(2) Changes in CBF velocity reflect relative changes
in CBF. Although correlation between absolute flow
velocity and CBF in any given population is poor,
good correlation exists between relative changes in
flow velocity and CBF.
Clinical applications of intraoperative TCD
monitoring
(1) Carotid endarterectomy
(a) Detection of ischemia. TCD monitoring during CEA
can detect cerebral ischemia during cross-clamping
of the carotid artery and allows selective shunting.
The correlation between changes in flow velocity and
in EEG appears to be excellent in many studies. A
decrease in Vmca of more than 60% compared to
preclamped baseline suggests inadequate CBF and the
need for an intraluminal shunt. TCD can also detect
malfunctioning shunts due to kinking or thrombosis.
(b) Detection of microemboli. Microemboli occur
frequently during CEA; embolic events may outweigh
hemodynamic events as an etiology of perioperative
stroke. TCD can detect both air and particulate
emboli.
(c) Diagnosis and treatment of postoperative hyperperfusion syndrome. Approximately 1% of
patients develop hyperperfusion syndrome following
CEA, resulting in cerebral hemorrhage. TCD
monitoring allows this diagnosis to be made and
treatment to be implemented. Patients who develop
the hyperperfusion syndrome show sustained elevation
of flow velocities after release of carotid
occlusion and often develop headaches. Prompt
reduction of blood pressure is effective in
normalizing the ipsilateral flow velocity and
alleviating symptoms.
(d) Diagnosis and treatment of postoperative intimal
flap or thrombosis. Occlusion of the carotid artery
postoperatively can occur due to clot formation or
the presence of an intimal flap. Sudden development
of clinical symptoms in the post-anesthesia care
unit should prompt an immediate TCD examination. A
prompt exploration may prevent an impending stroke.
(2) Cardiac surgery. Stroke as a complication of
cardiac surgical procedures occurs in up to 10% of
patients. More subtle neurologic and cognitive
dysfunction has been reported in 30% to 70% of
patients.
Adverse cerebral outcomes are due either to embolic
events or hypoperfusion, or both.
(a) Cerebral emboli during CPB. There is
considerable evidence to suggest that the delivery
of microemboli (air, platelet aggregate, and
thrombus) into the cerebral circulation is
responsible for the neuropsychologic deterioration
seen after bypass procedures. Patients with the
highest emboli counts have been observed to have
greater neurologic deterioration. Other studies have
shown that the presence of arterial line filters
reduces the incidence of emboli detected by TCD.
Despite these published findings, TCD monitoring of
emboli is still evolving and faces a number of
technical challenges.
(b) Cerebral perfusion during CPB. Brain injury
during CPB may also occur as a result of hypoperfusion. TCD has been used as a noninvasive
tool for examining CBF in patients having cardiac
surgical procedures performed under CPB. The
validity of TCD as a surrogate estimate of CBF
during CPB is unclear at present, however.
(3) Closed head injury. TCD can be used to assess
autoregulation and diagnose hyperemia and vasospasm
as well as intracranial circulatory arrest. With
elevation of ICP and cessation of CBF (intracranial
circulatory arrest), a characteristic oscillating
pattern is evident on TCD, with forward flow during
systole and backward flow during diastole. In this
context, although not widely used intraoperatively,
TCD may be useful in monitoring patients with head
injuries undergoing non-neurosurgical procedures.
(4) Diagnosis of brain death. When the oscillating
pattern is persistent and reproducible, the American
Academy of Neurology accepts it as a useful adjunct
for the confirmation of brain death.
Limitations of TCD as a routine intraoperative
monitor
(1) Most TCD monitors are designed for diagnostic
rather than monitoring purposes. A fixation device
is needed to allow
continuous, reliable recording that does not
interfere with the surgical procedure.
(2) Although most TCD equipment is fairly easy to
operate, skill and training are nevertheless
required.
(3) The successful transmission of ultrasound
through the skull depends on the thickness of the
skull, and the temporal bone thickness varies with
gender, race, and age. The failure rate can be
anywhere from 5% to 20%, depending on the patient
population.
ICP. Monitoring ICP allows optimization of CPP and
possibly prevents herniation. Monitoring methods
currently available include ventriculostomy,
subarachnoid bolt, epidural sensor, and fiberoptic
intraparenchymal monitor; the latter is the most
commonly used. The fiberoptic ICP monitors may allow
simultaneous measurement of brain temperature, a
function that is particularly useful in the ICU
where fever exacerbates secondary injury. In
addition, some incorporate LDF, partial pressure of
arterial oxygen (Pao2), partial pressure of arterial
carbon dioxide (Paco2), and pH monitoring.
Although its use is fairly common in the management
of patients with head injury in the ICU, the ICP
monitor is available only as an intraoperative
monitor when placed preoperatively by the
neurosurgeon.
III. Monitoring cerebral oxygenation and metabolism
Invasive monitoring
Brain tissue oxygenation (Po2). In the setting of
head injury, where secondary injury from
ischemia/hypoxia is of concern, maintaining a
reasonable CPP may not be adequate for brain
preservation. Significant inter-patient variability
exists with respect to the CPP below which brain
hypoxia is encountered. In this situation, a tissue
Po2 monitor is useful for assessing the balance
between oxygen supply and demand.
When a miniature Clarke-type polarographic electrode
originally designed for continuous intra-arterial
monitoring is used, the tissue Po2 monitor is placed intraparenchymally, typically in conjunction with an
ICP monitor. As such, the monitor reveals regional
or local, rather than global, oxygen levels. An
oxygen tension of 10 mm Hg is considered the
threshold for brain hypoxia. This value can be
increased either by increasing supply of oxygen
(supplemental O2, raising CPP, treating anemia) or
by decreasing demand (propofol or barbiturate
therapy). In addition to identifying inadequate
cerebral Po2, this monitor may demonstrate hyperoxia
that could occur with absolute or relative cerebral
hyperemia such as from loss of cerebral
autoregulation.
Some controversy exists regarding whether tissue Po2
monitors should be placed within a region of normal
brain parenchyma or adjacent to the injured brain
area.
Jugular bulb venous oximetry monitoring. In contrast
to the regional information that the tissue Po2
monitor provides, the jugular bulb oximeter allows
continuous or intermittent estimate of the global
balance between cerebral oxygen demand and supply.
Provided the cerebral metabolic rate for oxygen
consumption (CMRo2) remains constant, calculation of
the arteriovenous oxygen content allows estimation
of the relative CBF. Even if CMRo2 does not stay
constant, the arteriovenous oxygen content
difference always reflects the balance between the
brain's oxygen demand and supply. Because arterial
oxygenation is usually 100%, provided that
hematocrit remains constant, the jugular venous
oxygen saturation (Sjvo2) equally reflects this
balance. The physiological principle behind jugular
venous oximetry is shown in Figure-4. Thus,
intraoperative cerebral ischemia from inadequate
perfusion pressure or excessive hyperventilation can
be diagnosed readily. The influence of jugular
venous desaturation on prognosis in patients with a
head injury has been documented, and continuous
jugular venous oximetry has become a routine monitor
in many neurointensive care units. The thermistor-tipped
catheter also measures temperature approximating
that of the brain. Its major limitations include its
global nature and therefore inability to detect
focal cerebral ischemia and its invasive nature. Its
reliability during CPB is also controversial.
Figure-4. Physiologic principles of jugular venous
oximetry. |
CMRo2 |
= |
CBF x AVDo2
|
AVDo2 |
= |
CMRo2
CBF
|
AVDo2 |
= |
arterialO2
content (Cao2) - jugular venous O2
content (Cjvo2) |
|
= |
(Hgb x 1.39 x Sao2
+ 0.003 x Pao2) - (Hgb x 1.39 x
Sjvo2 + 0.003 x Pjvo2) |
|
= |
Hgb x 1.39 (Sao2
- Sjvo2) + 0.003 (Pao2
- Pjvo2) |
|
= |
Hgb x 1.39 (Sao2
- Sjvo2) (ignoring the
amount of dissolved O2) |
Interpretation of Sjvo2 values. The normal arterio-jugular
difference for O2 (AVDo2) value is 2.8 mcmol/mL or
6.3 vol% of oxygen. (Range 2.2 to 3.3 mcmol/mL, or 5
vol% to 7.5 vol%), and Sjvo2 is between 60% and 70%.
When oxygen delivery is higher than oxygen demand,
as in hyperemia, AVDo2 decreases and Sjvo2
increases. During periods of global cerebral
ischemia, AVDo2 widens and Sjvo2 decreases.
(1) Increased values. An Sjvo2 value of more than
90% indicates absolute or relative hyperemia. This
can occur as a result of a reduced metabolic need
(e.g., a comatose or brain-dead patient) or from
excessive flow (e.g., severe hypercapnia). Patients
with cerebral arteriovenous malformations have a
direct arterial shunt into the venous circulation
and thus have abnormally increased Sjvo2 values.
Extracranial contamination, either from the facial
vein or from rapid withdrawal in blood sampling
(intermittent measurement), also results in an
elevated value.
(2) Normal values. Although a normal balance between
flow and metabolism results in a normal Sjvo2, it
does not exclude the presence of focal ischemia.
Because the blood in the jugular veins drains all
areas of the brain, a discrete area of ischemia or
infarction might not influence the overall level of
saturation.
(3) Decreased values. On the other hand, Sjvo2 is
sensitive to global cerebral ischemia. A value of
<50% reflects increased O2 extraction and indicates
a potential risk of ischemic injury. This may be due
to increased metabolic demand, as in fever or
seizure, not matched by an equivalent increase in
flow, or due to an absolute reduction in flow. A
significant decrease in O2-carrying capacity due to
a decrease in hematocrit also leads to desaturation.
Ischemia may also alter CMRo2 measurements and
affect the interpretation of Sjvo2. As ischemia
progresses to infarction, oxygen consumption
decreases and Sjvo2 normalizes.
Microdialysis catheters. A small catheter, typically
inserted in conjunction with an ICP or tissue Po2
monitor, allows sampling of small molecules in the
interstitial fluid. The catheter, 0.6 mm in
diameter, has a 10-mm dialysis membrane. Artificial
cerebrospinal fluid (CSF) circulates through the
catheter, allowing equilibration with the
extracellular fluid and subsequent analysis of its
chemical
composition. This monitor's value is in determining
the metabolic state of the brain and assessing the
local cellular integrity. Three markers are thought
to be valuable for these purposes. An increasing
lactate/pyruvate ratio is sensitive to the onset of
ischemia. High levels of glycerol suggest inadequate
energy to maintain cellular integrity and the
resultant membrane breakdown. Finally, excitatory
amino acids, such as glutamate, are both a marker
for neuronal injury and a factor in its
exacerbation.
Currently, the microdialysis catheter is primarily
used in two situations: (a) extensive subarachnoid
hemorrhage where subsequent vasospasm is likely and
(b) traumatic brain injury (TBI) of sufficient
severity to warrant ICP/CPP monitoring. In either
situation, a significant risk exists for the
development of secondary injury due to cerebral
ischemia.
Location of the microdialysis catheter is important
because it provides information regarding only a
small surrounding region of brain. With subarachnoid
hemorrhage, the catheter should be placed in the
region of the brain perfused by the vessel most
likely at risk for vasospasm. Placement in TBI
depends on the nature of the injury. With diffuse
injury, the recommendation is to place the catheter
in the right frontal region. A focal contusion
warrants one catheter in the pericontusional area
but not within the contusion and a second catheter
in the normal brain.
Noninvasive monitoring. Near-infrared spectroscopy (NIRS)
or transcranial oximetry measures cerebral regional
oxygen saturation by measuring near-infrared light
reflected off the chromophobes in the brain, the
most important of which are oxyhemoglobin,
deoxyhemoglobin, and cytochrome A3. NIRS has been
studied in a variety of clinical settings including
CEA and hypothermic circulatory arrest. Its major
limitations include the intersubject variability,
the variable length of the optical path, the
potential contamination from extracranial blood, and
most important, the lack of a definable threshold.
Because of the thin scalp and skull in the neonate
and infant, NIRS holds promise in this patient
population but remains an investigative tool in its
present form.

|