To understand the effects of anesthesia and
surgery on the nervous system, one needs
to know basic cellular neurophysiology
as well as organ-level physiologic
function.
Cellular
neurophysiology
The basic properties of neuronal
excitability are due to a change in the
membrane potential so that a threshold
is reached and the neuron fires an
action potential. This propagates to the
axon terminal and releases a
neurotransmitter that influences the
membrane potential of a second neuron.
Membrane potentials are voltages
measured across the cell membrane due to
an unequal distribution of ions across
that membrane. A combination of the
equilibrium potential for a particular
ion and the membrane's conductance
(permeability) for that ion determines
its contribution to the membrane
potential.
The equilibrium potential (E) for an ion
can be calculated using the Nernst
equation if the intra- (for potassium, Ki)
and extracellular (K0)
concentrations of that ion are known.
For an ion with a single positive
charge, the equation simplifies to EK
= -61 log [Ki/K0]
at 37°C. Under normal conditions in the
nervous system, the equilibrium
potential for potassium (K) is
approximately -90 mV and for sodium (Na)
+45 mV.
(1) The relative conductance of the
neuronal membrane to different ions
determines the membrane potential. This
conductance (g) for the different ions
varies with conditions, input to the
specific neuron, and time. The membrane
potential of a neuron at any point in
time can be described by the following
equation:
Em
= [gk(Ek)+gNa(ENa)+gx(Ex)]/
gK +gNa+gx
where gx is the
conductance for ion x and Ex
is the equilibrium potential for that
ion. The resting membrane potential for
a neuron is approximately -70 mV, which
is closer to the Ek
(-90 mV) than the ENa
(+45 mV) because gK is much greater than
gNa in resting
(unexcited) neurons.
(2) There are concentration-dependent
and electrical field-dependent forces
acting on ions; the sum of these forces
determines whether the net movement of a
particular ion will be into or out of
that neuron. This is referred to as the
electrochemical gradient for that ion.
Action potentials are regenerative
changes in a neuron's membrane potential
due to excitation of the neuron so that
its membrane potential depolarizes past
a certain threshold. During an action
potential, a rapid initial increase in
the gNa is followed by
a return to baseline and a slower
increase in the gK.
These conductance changes lead to a
short and rapid depolarization followed
by a repolarization. This is sometimes
followed by a hyperpolarization after
the action potential (Figure 1).
The Na conductance changes are due to
the opening of a protein channel in the
membrane that is selectively permeable
to Na ions. This channel has one
activation and one inactivation gate,
both of which must be in the open
configuration if the channel is to allow
Na through it. The rapid opening and
closing of this channel are in part
responsible for the brief duration of
the action potential.
At rest, more K than Na channels are
open. With the action potential, more Na
channels open so that the gNa
is greater than the gK
and the neurons depolarize. The
depolarization causes a slow opening of
K channels, increasing gK
and leading to a repolarization (gK
> gNa). In the period
after the action potential, when the Na
channels have become inactivated, the
increased gK can actually cause a
hyperpolarization below the resting
potential; this so-called
afterhyperpolarization is frequently
found in neurons.
(3) Synaptic transmission is the process
by which one neuron (presynaptic neuron)
influences the membrane potential and
thereby the action potential generation
in a second neuron (postsynaptic
neuron). The axon terminals of a neuron
contain vesicles with neurotransmitter
molecules in them. When a terminal is
depolarized, voltage-sensitive calcium
(Ca) channels open, increasing the Ca
concentration in the terminal. This Ca
increase causes the vesicles to release
a neurotransmitter into the synaptic
cleft. The transmitter diffuses across
the synapse and binds to a specific
receptor on the postsynaptic neuron. Its
effect on the postsynaptic neuron
depends on ion channels that are opened
or biochemical processes that are
altered by the activation of that
receptor.
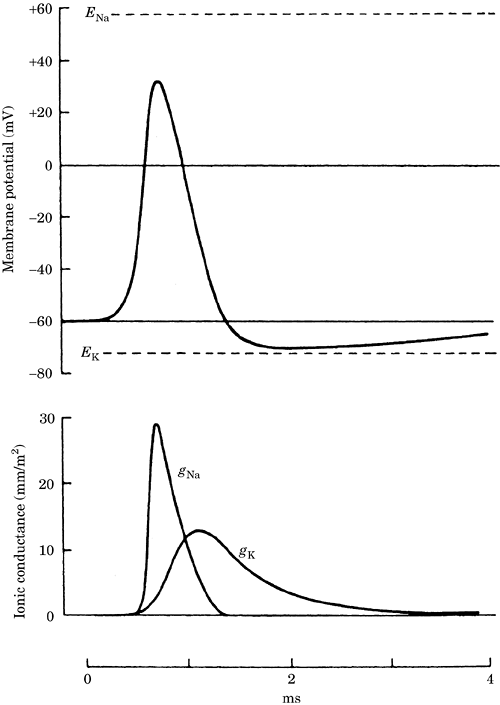
Figure 1. Changes in the membrane
potential and the sodium and potassium
conductances (gNa and
gK) during an action
potential. ENa and
Ek are the sodium and
potassium equilibrium potentials.
Ionotropic receptor activation opens
membrane channels for certain ions that
can either hyperpolarize or depolarize
the postsynaptic neuron, making it less
or more likely to fire an action
potential.
Metabotropic receptors can activate
second messengers that alter neuronal
biochemical parameters. This can effect
long-term changes in a neuron's
activity.
(4) Glutamate is a major excitatory
neurotransmitter in the central nervous
system (CNS). Its activation depolarizes
neurons, increasing the number of action
potentials generated.
There are three major ionotropic
glutamate receptors:
alpha-amino-3-hydroxy-5-methyl-4-isoxazole
propionic acid (AMPA), kainate, and
N-methyl-D-aspartic acid (NMDA). The
AMPA and kainate receptors are attached
to ion channels that allow Na and K to
pass through them; a small number of
AMPA receptors are also permeable to Ca.
The NMDA channels activated when neurons
are already depolarized are permeable to
Na, K, and Ca. Activation of NMDA
channels has been associated with
long-term changes in neuronal activity
that may be cellular correlates of
learning and memory. Overactivation of
glutamate receptors has been associated
with neuronal injury from epilepsy,
trauma, and ischemia.
Metabotropic receptors are also
activated by glutamate. These receptors
act via guanosine-triphosphate (GTP)-binding
proteins (G proteins) to affect ion
channels or second-messenger pathways
(e.g., 3'-5'-cyclic-adenosine monophosphate [cAMP], inositol
1,4,5-trisphosphate [IP3]), which in
turn can alter ionic conductance, cell
Ca levels, and a host of other
biochemical changes. The effect of
metabotropic receptor activation is
longer in duration than that of
inotropic receptor activation.
(5) Gamma-aminobutyric acid (GABA) and
glycine are major inhibitory
neurotransmitters in the CNS. Their
activation hyperpolarizes neurons,
decreasing the number of action
potentials generated. Inhibition is
important for the brain and spinal cord
to function. When inhibition is
substantially reduced, seizures can
occur and lead to complete loss of
function and permanent brain damage.
GABA is a major inhibitory transmitter
in the brain and spinal cord. The GABAA
receptor contains a chloride channel
that is opened when GABA binds. This
activity is augmented by
benzodiazepines, volatile anesthetics,
and barbiturates. The GABAB receptor
acts via a second messenger to open K
channels.
Glycine is a major inhibitory
transmitter in the spinal cord.
Strychnine blocks the action of glycine.
Active transport maintains the ionic
concentrations required for neuronal
function. There is a constant leak of
ions down their electrochemical
gradients. If not corrected, this leak
leads to a loss of these ion gradients.
Ion pumps use energy to maintain the ion
concentrations necessary for neuronal
viability. During ischemia, a decrease
in energy production and a loss of ion
gradients occurs (Figure-2).
Adenosine triphosphate (ATP), neuronal
is a source of energy for many ion
pumps. The Na/K ATPase pump maintains
high intracellular K concentrations and
low intracellular Na concentrations. The
pump compensates for the leak of these
ions in inactive neurons and the large
changes in these ions during the action
potential. If this pump is blocked,
neurons quickly lose their ability to
function. ATPase pumps in the plasma
membrane and the endoplasmic reticulum
maintain low cytosolic Ca concentrations
in neurons.
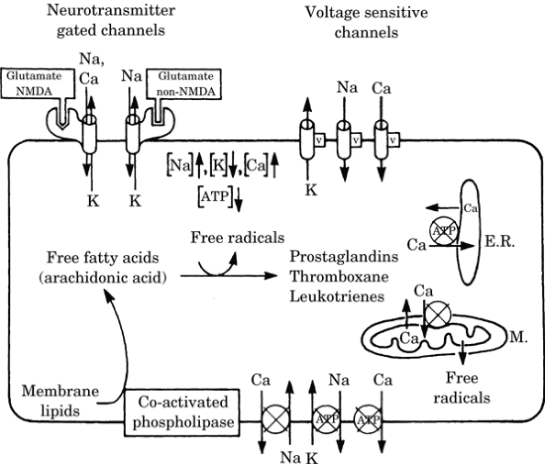
Figure-2. Effect of ischemia on ion and
metabolite levels in neurons. For
clarity, ion channels are shown on the
top membrane and ion pumps on the bottom
membrane; their actual location can be
on any membrane surface. Circles
indicate energy-driven pumps; an x
through the circle indicates that this
pump is blocked or has reduced activity
during ischemia. V indicates a
voltage-dependent channel. NMDA,
N-methyl-D-aspartic acid; ATP, adenosine
triphosphate.
The Na gradient is a source of energy
for ion pumps and amino acid
transporters. These active transporters
couple the energy of Na as it goes down
its electrochemical gradient with the
pumping of other ions and metabolites up
their gradients. To maintain appropriate
cellular levels of Ca and hydrogen (H),
Na/Ca and Na/H exchangers are
important transporters. The transport of
glutamate and other amino acids from the
extracellular to the intracellular
compartment also uses the energy of the
Na gradient. The gradient is maintained
by the Na/K ATPase pump; thus, the
ultimate energy for this ion exchanger
comes from ATP used to power the Na/K
pump.
When energy fails due to hypoxia or
ischemia, the pumps can no longer
maintain the gradients, intra- and
extracellular ion concentrations change
and the neurons depolarize, leading to a
rapid and complete membrane
depolarization and eventual cell death.
This is illustrated in Figure-2.
Regional
neurophysiology. Different
regions of the brain subserve different
and distinct functions.
(1) The primary somatosensory cortex
located on the postcentral gyrus is the
cortical locus where somatic sensations
converge. Association areas that aid in
the interpretation of these sensations
are located posterior to this gyrus.
(2) The primary motor cortex is located
on the precentral gyrus and has output
to motor neurons in the spinal cord.
Premotor association areas are located
anterior to this gyrus and receive input
from other important motor centers of
the brain including the cerebellum, the
basal ganglia, and the red nucleus. The
reticular formation also has important
motor functions.
The primary visual and visual
association areas are located in the
occipital lobe.
(4) The primary auditory and auditory
association areas are located in the
temporal lobe.
(5) Wernicke's area is located on the
angular gyrus in the dominant
hemisphere. It is a multimodal
association area. Lesions in this area
are devastating and can lead to the loss
of comprehension of written and spoken
words.
(6) The frontal association areas are
important for controlling personality
and directing intellectual activity
through sequential steps toward a goal.
(7) The limbic areas of the brain, are
located medially. Limbic system
structures include the hypothalamus, the
amygdala, the hippocampus, and the
limbic cortex. These areas are
associated with feelings of reward and
punishment, emotional behavior,
learning, and memory. The hippocampus is
essential for the transformation of
short-term to long-term memory. The
hypothalamus controls many bodily
vegetative functions (cardiovascular,
temperature, and water regulation).
(8) The brain stem contains the
reticular activating system, which is
responsible for maintaining alertness.
The vasomotor areas located in the brain
stem are important for circulatory
control. Lesions in the brain stem can
lead to coma.
(9) The spinal cord is important as a
pathway for information between the body
and the brain as well as for the
generation of certain reflexes. Input to
the spinal cord comes via the dorsal
root to the dorsal horn; output from
motor neurons, which are located in the
ventral horn, is via the ventral root.
Input to the brain via the spinal cord
can be modified before transmission to
the brain via ascending tracts. Indeed,
descending pathways can reduce pain
input at the spinal level. These
pathways are activated by periaqueductal
and periventricular gray regions of the
brain.