| Postoperative
Complications |
Respiratory Care|
Cardiovascular Therapy|
Fluid Management| Nutrition in Critical Patients
|Traumatic Brain Injury-Stroke-Brain Death
|

The fluid management in patients
who have central nervous system (CNS) pathology
presents special challenges for anesthesiologists
and intensivists. These patients often receive
diuretics (e.g., mannitol, furosemide) to treat
cerebral edema and to reduce intracranial
hypertension. At the same time, neurosurgical
patients may require large volumes of either
intravenous fluid or blood as part of an initial
resuscitation, treatment of cerebral vasospasm,
correction of preoperative dehydration, or
maintenance of intraoperative and postoperative
hemodynamic stability.
Fluid restriction had been a cornerstone of the
treatment of patients with CNS pathology for many
years. This practice arose from the concern that the
administration of fluid might exacerbate cerebral
edema and intracranial hypertension. Not only has
the efficacy of fluid restriction remained unproven,
even after all this time, but also the consequences
of fluid restriction, if pursued to the point of
hypovolemia, can be devastating. A recent study of
head trauma patients demonstrated that negative
fluid balance (<594 mL) was associated with an
adverse effect on outcome, independent of its
relationship to intracranial pressure (ICP), mean
arterial pressure, or cerebral perfusion pressure.
Although few human data exist concerning the impact
of exogenous fluids on the injured brain to guide
rational fluid management in the neurosurgical
patient, it is possible to examine the factors that
influence water movement into the brain and make
some reasonable recommendations.
I.
Osmolality/osmolarity, colloid oncotic pressure
(COP), crystalloid, and colloid
Osmotic pressure.
This is the hydrostatic force acting to equalize the
concentration of water (H2O) on both sides of a
membrane that is impermeable to substances dissolved
in that water. Water moves along its concentration
gradient. This means that if a solution containing
10 mosmol of sodium (Na) and 10 mosmol of chloride
(Cl) are placed on one side of a semipermeable
membrane with H2O on the other, water
will move "toward" the saline (NaCl) solution. The
saline solution has a concentration of 20 mosmol/L,
and the force driving water will be approximately
19.3 mm Hg/mosmol, or 386 mm Hg. Note that the
driving force is proportional to the gradient across
the membrane; if two solutions of equal
concentration are placed across a membrane, there is
no driving force. Similarly, if the membrane is
permeable to the solutes (e.g., Na and Cl), this
reduces the gradient and hence the osmotic forces.
Osmolarity and osmolality.
Osmolarity describes the molar number of osmotically
active particles per liter of solution. In practice,
this value is typically calculated by adding up the
milliequivalent (mEq) concentrations of the various
ions in the solution. Osmolality describes the molar
number of osmotically active particles per kilogram
of solvent. This value is directly measured by
determining either the freezing point or the vapor
pressure of the solution (each of which is reduced
by a dissolved solute). Note that osmotic activity
of a solution demands that particles be
"independent." As NaCl dissociates into Na and Cl,
it creates two osmotically active particles. If
electrostatic forces act to prevent dissociation of
the two charged particles, osmolality is reduced.
For most dilute salt solutions, osmolality is equal
to or slightly less than osmolarity. For example,
commercial lactated Ringer's solution has a
calculated osmolarity of approximately 275 mosmol/L
but a measured osmolality of approximately 254
mosmol/kg, indicating incomplete dissociation.
Calculated versus measured serum osmolality is
relevant in the clinical setting. The reference
method for measuring serum osmolality is the
delta-cryoscopic technique. However, the technology
may not be available, and/or it is not always
possible to obtain an emergency measurement 24 hours
a day. In such cases, osmolality can be calculated
from the osmoles that are routinely measured, such
as sodium, potassium, urea, and glucose. Be advised,
however, that calculation of osmolality introduces a
bias, overestimating osmolality in the lower ranges
and underestimating it in the higher ranges.
COP. Osmolarity
and osmolality are determined by the total number of
dissolved "particles" in a solution regardless of
their size. COP is the osmotic pressure produced by
large molecules (e.g., albumin, hetastarch,
dextran). This factor becomes particularly important
in biological systems in which vascular membranes
are often permeable to small ions but not to large
molecules (typically plasma proteins). In such
situations, proteins might be the only osmotically
active particles. Normal COP is approximately 20 mm
Hg (or equal to approximately 1 mosmol/kg).
Starling's hypothesis.
In 1898, Starling published his equations describing
the forces driving water across vascular membranes.
The major factors that control the movement of
fluids between the intravascular and extravascular
spaces are the transcapillary hydrostatic gradient,
the osmotic and oncotic gradients, and the relative
permeability of the capillary membranes that
separate these spaces. The Starling equation is as
follows:
FM = k (Pc + π - Pi - πc)
where FM is fluid movement, k is the filtration
coefficient of the capillary wall (i.e., how leaky
it is), Pc is the hydrostatic pressure in the
capillaries, Pi is the hydrostatic pressure (usually
negative) in the interstitial (extravascular) space,
and πi and πc are interstitial and capillary
osmotic pressures, respectively.
Fluid movement is therefore proportional to the
hydrostatic pressure gradient minus the osmotic
pressure gradient across a vessel wall. The
magnitude of the osmotic gradient depends on the
relative permeability of the vessels to solute. In
the periphery (muscle, bowel, lung, etc.), the
capillary endothelium has a pore size of 65 Å and
is freely permeable to small molecules and ions (Na,
Cl) but not to large molecules, such as proteins
(Fig.-1). As a result, π is defined only by
colloids, and the Starling equation can be
simplified by saying that fluid moves into a tissue
whenever either the hydrostatic gradient increases
(either intravascular pressure rises or interstitial
pressure falls) or the osmotic gradient decreases.
In normal situations, the intravascular protein
concentration is higher than the interstitial
concentration, acting to draw water back into the
vascular space. If COP is reduced (e.g., by dilution
with large amounts of isotonic crystalloid), fluid
begins to accumulate in the interstitium, producing
edema. This fact is familiar to all
anesthesiologists who have seen marked peripheral
edema in patients given many liters of crystalloid
during surgery or resuscitation. By contrast, the
blood-brain barrier (BBB) is impermeable to both
ions and proteins so that osmotic pressure is
determined by the total osmotic gradient, of which
COP contributes only a tiny fraction.
Figure-1 Schematic
diagram of a peripheral capillary. |
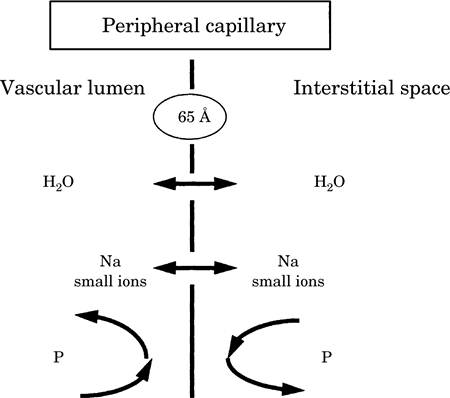 |
The vessel wall is
permeable to both water (H2O) and
small ions but not to proteins (P) |
Interstitial clearance. Peripheral tissues have a
net outward movement of fluid (i.e., the value of FM
is positive). Edema is not normally present,
however, because this extravasated fluid is cleared
by the lymphatics. While many researchers agree that
there is some lymphatic drainage of the brain, most
interstitial fluid in the brain is cleared either by
bulk fluid flow into the cerebrospinal fluid (CSF)
spaces or via pinocytosis back into the
intravascular compartment. This is a slow process
and probably does not counteract rapid fluid
movement into the interstitial space.
Hydrostatic forces and interstitial compliance. In
the tissues, the net hydrostatic gradient is
determined by (a) intravascular pressure and (b)
interstitial tissue compliance. Normally, the
direction is outward (capillary to interstitium).
There is no question that in the brain (or in any
organ), elevated intravascular pressure, such as
that produced by either high jugular venous pressure
or a head-down posture, can increase edema
formation. However, an often overlooked factor that
influences the pressure gradient is the interstitial
compliance (i.e., the tendency of tissue to resist
fluid influx). The loose interstitial space in most
peripheral tissues does little to impede the influx
of fluid. This explains the ease with which edema
develops around, for example, the face and the eyes,
even with minor hydrostatic stresses (e.g., a
facedown posture).
By contrast, the interstitial space of the brain is
extremely noncompliant, resisting fluid movement. As
a result, minor changes in driving forces (either
hydrostatic or osmotic/oncotic) do not produce
measurable edema. However, a vicious cycle can
develop so that, as edema forms in the brain, the
interstitial matrix is disrupted, the compliance
increases, and additional edema forms more easily.
In contrast, the closed cranium and ICP can act to
retard fluid influx. This may partially explain the
exacerbation of edema formation that can occur after
rapid decompression of the intracranial space.
Can we explain the influence of certain fluids on
the brain? In contrast to capillaries elsewhere in
the body, the endothelial cells in the brain are
held together by continuous tight junctions to form
the BBB. There are no intercellular gaps: the
membranes are not fenestrated and do not have
channels or chains of vesicles that form transendothelial pathways. The effective pore size
of the BBB is only 7 to 9 , making this unique
structure normally impermeable to large molecules
(e.g., plasma proteins and synthetic colloids, such
as hetastarch and dextrans) and relatively
impermeable to many small polar solutes (Na, K, Cl)
(Fig.-2). The BBB functions as a semipermeable
membrane that allows only water to move freely
between the brain's interstitial space and the
vasculature. This should serve to make the brain an
exquisitely sensitive osmometer where water moves
according to osmotic gradients.
Figure-2. Schematic
diagram of a cerebral capillary. |
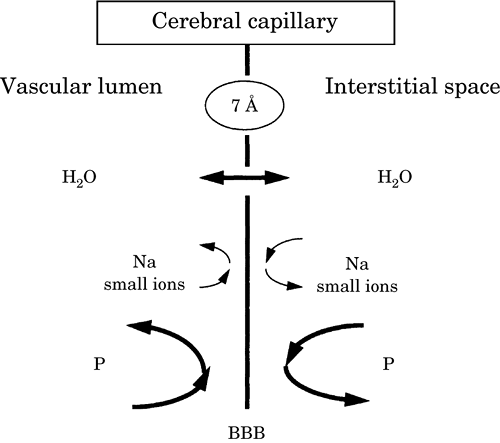 |
The blood-brain barrier (BBB) is
impermeable to small ions and proteins (P) but not
to water (H2O) |
We would then predict that reducing serum osmolality
(e.g., by infusing water or large volumes of
nonisotonic crystalloid solution) increases cerebral
edema; conversely, increasing osmolality reduces
brain water content. This experiment was first done
in 1919 by Weed and McKibben, who showed a large and
rapid increase in brain volume with the reduction of
serum osmolality. Since that time, numerous studies
have demonstrated the exquisite sensitivity of the
brain's water content to changes in serum
osmolality, in which even small changes can produce
measurable changes in the brain water content. This
makes sense considering that a 5 mosmol/kg gradient
is equivalent to an almost 100 mm Hg force driving
water (see preceding text). In fact, in experimental
animals, a reduction in plasma osmolality of as
little as 5% under otherwise normal conditions
causes brain edema and increases ICP.
What about changes in COP?
As mentioned, colloidally
active molecules contribute to only a tiny fraction
of the total osmolality and, when the BBB is intact,
can be responsible for only a small driving force.
Normal plasma COP is approximately 20 mm Hg, whereas
that in the brain interstitium is approximately 0.6
mm Hg. This is equal to the force that could be
generated by a change in the capillary/tissue
osmotic gradient of only 1 mosmol/kg. We would
therefore predict that changes in COP have only
minimal effect on brain water content. This
hypothesis has been tested directly in several
animal experiments, which have demonstrated that
normal brain water content can be altered by small
changes in osmolality but not by clinically
achievable changes in COP. There was also no
increase in brain water content (i.e., edema) in any
region with an intact BBB, even after a very large
reduction (approximately 50%) in COP.
However, what about more clinically relevant
conditions, with varying degrees of BBB abnormality?
We can predict that if the BBB is made completely
permeable to both small and large molecules (i.e.,
complete breakdown of the BBB as is common with
several experimental and clinical injuries), it
should be impossible to maintain any form of osmotic
or oncotic gradient between the intravascular
compartment and the brain interstitium. As a result,
no changes in brain water content would be expected
with a change in either gradient. Indeed, in several
animal models resembling human brain injuries (e.g.,
implanted glioma and freezing lesion, which are
similar to brain tumor and trauma, respectively),
changes induced by a reduction in total serum
osmolality were seen only in apparently normal
regions of the brain relatively distant from the
focus of injury. In keeping with this, several
studies have shown that acute hyperosmolality (as
with mannitol, urea, glycerol, hypertonic saline
[HS]) reduces water content only in relatively
normal brain tissue where the BBB is intact.
Conversely, when the BBB is severely damaged,
investigations have failed to demonstrate that
reducing the COP affects cerebral edema. In the
presence of mild injury to the BBB in experimental
animals, a reduction in COP may potentially
aggravate brain edema. Therefore, it is possible
that, with a less severe injury, the BBB may
function similarly to the peripheral tissue.
Summary. Injury to the brain interferes with the
integrity of the BBB to varying degrees, depending
on the severity of the damage. Regions in which
there is a complete breakdown of the BBB, there will
be no osmotic/oncotic gradient. Water accumulation
(i.e., brain edema) occurs because of the pathologic
process and cannot be directly influenced by the
osmotic/oncotic gradient. In other regions where
there is moderate injury to the BBB (i.e., a mild
opening rendering pore size similar to the
periphery), it is possible that the colloid oncotic
gradient is effective as in the peripheral tissue.
Finally, the BBB is normal in a significant portion
of the brain. The presence of a functionally intact
BBB is essential if osmotherapy is to be effective.
Fluids for intravenous administration.
Table-1
lists a variety of solutions suitable for
intravenous use.
Table-1. Composition of intravenous fluids |
Fluid |
Dextrose (g/L) |
Na (mEq/L) |
Cl (mEq/L) |
Osmolaritya
(mosmol/L) |
Oncotic P (mm Hg) |
5% Dextrose in
water |
50 |
- |
- |
278 |
- |
Crystalloids: |
5% Dextrose in
0.45% saline |
50
|
77
|
77
|
405 |
- |
5% Dextrose in
Ringer's |
50
|
130
|
109
|
525 |
- |
Lactated Ringer's
|
|
130 |
109
|
275 |
- |
Plasmalyte |
|
140
|
98
|
298 |
- |
0.45% Saline |
|
77
|
77
|
154 |
- |
0.9% NS |
|
154
|
154
|
308 |
- |
3.0% Saline |
|
513
|
513
|
1026 |
- |
5.0% Saline |
|
855
|
855
|
1711 |
- |
7.5% Saline |
|
1283
|
1283
|
2567 |
- |
20% Mannitol |
|
- |
- |
1098 |
- |
Colloids:
|
Plasma |
|
|
|
295
|
21 |
Albumin
(5%) |
|
|
|
290 |
19 |
Hetastarch (6%) in
NS |
|
154
|
154
|
~310
|
31 |
Dextran (10%) 40 in
NS |
|
154
|
154
|
~310
|
169 |
Dextran (6%) 70 in
NS |
|
154
|
154
|
~310
|
19 |
aOsmolarity = calculated value (mosmol/L = mg ÷
molecular weight × 10 × valence). NS, normal
saline; —, no available data; P, pressure. |
Crystalloid is the term commonly applied to
solutions that do not contain any high molecular
weight compound and have an oncotic pressure of
zero. Crystalloids can be hyposmolar, iso-osmolar,
or hyperosmolar, and may or may not contain glucose.
Crystalloids can be made hyperosmolar by the
inclusion of electrolytes (e.g., Na and Cl, as in
HS) and low molecular weight solutes such as
mannitol (molecular weight 182) or glucose
(molecular weight 180).
Colloid is the term used to denote solutions that
have an oncotic pressure similar to that of plasma.
Some commonly administered colloids include 5% or
25% albumin, plasma, 6% hetastarch (hydroxyethyl
starch, molecular weight 450), pentastarch (a low
molecular weight hydroxyethyl starch, molecular
weight 264), and the dextrans (molecular weights 40
and 70). Dextran and hetastarch are dissolved in
normal saline so that the osmolarity of the solution
is approximately 290 to 310 mosmol/L with a sodium
and chloride content of approximately 154 mEq/L,
which makes them hyperoncotic and hypertonic.
Hetastarch should be limited and used with caution,
however, because of the depletion of factor VIII and
possible coagulation difficulties encountered with
volumes exceeding 1,500 mL. Dextran-40 interferes
with normal platelet function and is therefore not
advisable for patients who have intracranial
pathology other than to improve rheology as in
ischemic cerebrovascular diseases. Although small
volumes of hetastarch and dextran can restore
normovolemia rapidly and without increasing ICP, it
is unclear whether they offer any advantage over the
combination of isotonic fluids and osmotic
diuretics.
II. Clinical fluid management of neurosurgical
patients
Fluid restriction. Despite the lack of convincing
experimental evidence that iso-osmolar crystalloids
are detrimental, fluid restriction is widely
practiced in patients who have mass lesions or
cerebral edema or are at risk for intracranial
hypertension. The only directly applicable data
indicate that clinically acceptable restriction has
little effect on edema formation. However, "modest"
fluid restriction has some logic. One of the few
human studies on fluid therapy in neurosurgical
patients has demonstrated that patients given
standard "maintenance" amounts of intravenous
fluid (e.g., 2,000 mL/day of 0.45 normal saline in
5% dextrose) in the postoperative period develop a
progressive reduction in serum osmolality (Fig.-3).
On the other hand, patients given half of this
volume over a period of several days (about a week)
show a progressive increase in serum osmolality,
which could account for dehydration of the brain
(Fig. 20-3). While no CNS parameters were measured,
the results suggest that usual maintenance fluids
contain excess free water for the typical
postoperative craniotomy patient. In this regard,
fluid restriction can be viewed as "preventing" hyposmotically driven edema. However, this does not
imply that even greater degrees of fluid restriction
are beneficial or that the administration of a fluid
mixture that does not reduce osmolality is
detrimental.
Figure-3. Effect of fluid
restriction (1 L/day) on serum osmolality in
neurosurgical patients. |
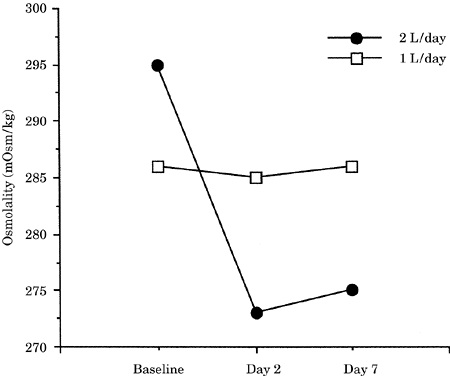 |
Intraoperative volume replacement/resuscitation. As
a general rule, intraoperative fluids should be
given at a rate sufficient to replace the urinary
output and insensible losses (e.g., skin and lungs).
Table-2 illustrates the intravascular volume
expansion obtained with different types of fluids.
Volume replacement with crystalloid solutions,
geared to maintain the hematocrit at approximately
33%, is calculated on a 3:1 ratio (crystalloid to
intraoperative blood loss) because of the larger
distribution space of the crystalloids.
Table-2. Volume replacement
|
Fluid Infused |
Intravascular Volume Increase
|
1 L isotonic
crystalloid |
~250 mL
|
1 L 5% albumin |
~500 mL |
1 L hetastarch |
~750 mL
|
The available data indicate that intravascular
volume replacement and expansion will have no effect
on cerebral edema as long as normal serum osmolality
is maintained and cerebral hydrostatic pressure is
not markedly increased (e.g., owing to true volume
overload and elevated right heart pressures).
Whether this is achieved with crystalloid or colloid
seems irrelevant, although the osmolality of the
selected fluid is crucial. With respect to this
issue, it should also be noted that lactated
Ringer's solution is not strictly isosmotic
(measured osmolality 252 to 255 mosmol/kg),
particularly when administered to patients whose
baseline osmolality has been increased by either
fluid restriction or hyperosmolar fluids (mannitol,
HS, etc.).
It is recommended that serum osmolality be
checked repeatedly with the goal of either
maintaining this value or increasing it slightly.
Fluid administration that results in a reduction in
osmolality should be avoided. Small volumes of
lactated Ringer's (1 to 3 L) are unlikely to be
detrimental and can be used safely, for example, to
compensate for the changes in venous capacitance
that typically accompany the induction of
anesthesia. If large volumes are needed (either to
replace blood loss or to compensate for some other
source of volume loss), a change to a more isotonic
fluid such as normal saline is probably advisable.
It is also important to remember that rapid infusion
of a large volume of 0.9% NaCl can induce a
dose-dependent hyperchloremic metabolic acidosis.
Whether this acid-base abnormality is, in fact,
harmful remains unclear, although animal studies
suggest that hyperchloremia causes renal
vasoconstriction. If large volumes are needed, a
combination of isotonic crystalloids and colloids
may be the best choice (Table 20-1).
These recommendations should not be interpreted,
however, as a license to "give all the isotonic
fluid you like." Volume overload can have
detrimental effects on ICP by increasing either
cerebral blood volume (CBV) or hydrostatically
driven cerebral edema formation.
Postoperative period. In the postoperative period,
the patient no longer needs large volumes of fluid.
The recommendation of Shenkin to give approximately
1,000 mL/day is probably reasonable (Fig. 20-3); we
would again recommend periodic measurement of serum
osmolality, particularly if the patient's neurologic
status deteriorates. If cerebral edema does develop,
further fluid restriction is unlikely to be of value
and can cause hypovolemia. Instead, treatment
consists of mannitol or furosemide and maintenance
of normovolemia with fluids to sustain the increased
osmolality. Inducing hypovolemia so that
vasopressors are required to maintain acceptable
hemodynamic parameters has little advantage (and
some disadvantage).
HS solutions. HS solutions have been evaluated for
use in fluid resuscitation since the 1960s,
particularly because hemodynamic improvement can be
achieved with very small volumes given very quickly.
Because hyperosmolality is known to reduce brain
volume, HS has been used in patients who are at risk
for increased ICP. In humans, acute resuscitation
from hemorrhagic shock with 7.5% HS is associated
with improved outcome in patients with multiple
trauma and head injuries. Clinical studies suggest
that HS may be better in hypotensive, brain-injured
patients during transportation to the hospital.
There is no question that HS can quickly restore
intravascular volume while reducing ICP through
brain water reduction in uninjured brain. Additional
benefit may be derived from decreases in CSF
production. Unfortunately, what remains unclear is
whether this approach is unique or whether the
identical CNS benefit could be achieved with any
resuscitation fluid that increases osmolality.
The principal disadvantage of HS is the danger of
hypernatremia. In a recent study of neurosurgical
patients undergoing elective supratentorial
procedures, we have shown that equal volumes of 20%
mannitol and 7.5% HS reduce brain bulk and CSF
pressure to the same extent (Fig.-3). However,
serum sodium increased during the administration of
HS and peaked at more than 150 mEq/L at the
conclusion of the infusion.
Mannitol and furosemide. Both mannitol and
furosemide (and occasionally other diuretics) are
used to control ICP and brain swelling in
neurosurgical patients. Mannitol acts by
establishing an osmotic gradient between blood and
brain in the presence of a relatively intact BBB.
This promotes removal of water from areas of normal
brain. Mannitol might elevate ICP transiently from
the vasodilator effects of hyperosmolality,
resulting in an increase in CBV. In both dogs and
humans, this phenomenon occurs neither in the
presence of intracranial hypertension nor when
mannitol is given at moderate rates. Mannitol may,
therefore, be given to most neurosurgical patients.
The exception might be patients who have significant
cardiovascular disease in whom the transient volume
expansion might precipitate congestive heart
failure.
The other important concern is that the repeated use
of mannitol may cause excessive hyperosmolality,
which can be deadly. In addition, mannitol
accumulates in the interstitium with repeated doses.
If interstitial osmolality rises excessively, it is
possible for the normal brain-blood gradient to be
reversed with resultant exacerbation of the edema.
Furthermore, if brain osmolality is increased, edema
may be enhanced by the subsequent normalization of
serum osmolality. The recommended dose of mannitol
is therefore 0.25 to 1 g/kg. The smallest possible
dose is selected and infused over 10 to 15 minutes.
The exact mechanism of action of furosemide remains
controversial, although it certainly is related to
the drug's ability to block Cl transportation.
Furosemide and similar drugs might also act
primarily by reducing cellular swelling rather than
by changing the extracellular fluid volume. Several
studies have demonstrated that furosemide decreases
CSF production. This effect can explain the
synergistic effect of mannitol and furosemide on
intracranial compliance. However, the maximal effect
of furosemide compared with that of mannitol is
delayed. For this reason, mannitol probably remains
the drug of choice for rapid control of ICP.
Glucose-containing solutions. Salt-free solutions
containing glucose should be avoided in patients who
have intracranial pathology. Free water reduces
serum osmolality and increases brain water content.
Furthermore, solid evidence in animals and humans
indicates that excessive glucose exacerbates
neurologic damage and can worsen the outcome from
both focal and global ischemia. This happens because
glucose metabolism enhances tissue acidosis in
ischemic areas. The reduction of adenosine levels
from hyperglycemia could also be detrimental.
Adenosine inhibits the release of excitatory amino
acids, which play a major role in ischemic cell
damage.
Although clinical investigations have indicated a
negative relationship between patients' plasma
glucose on admission and outcome after stroke,
cardiac arrest, and head injury, more recent studies
suggest that this correlation is not necessarily one
of cause and effect because the high glucose may be
a concomitant of more severe CNS damage. Nor has it
been possible to demonstrate that the administration
of glucose to humans is detrimental. Nevertheless,
withholding glucose from adult neurosurgical
patients is not associated with hypoglycemia. It may
therefore be prudent to withhold glucose-containing
fluids from acutely injured and elective surgical
patients. This caveat does not apply to the use of
hyperalimentation in such patients, perhaps because
the administration of these hyperglycemic solutions
typically begins several days after the primary
insult.
Should insulin be administered to correct
hyperglycemia in patients who have intracranial
pathology? While laboratory evidence suggests that
the correction of hyperglycemia with insulin before
the ischemic insult occurs improves outcome, this
has not been studied in humans.
Summary. Blood sugar in neurosurgical patients
should be controlled carefully to avoid both hypo-
and hyperglycemia and to maintain glucose between
100 and 150 mg/dL. Glucose-containing solutions
should be withheld, except in the case of neonates
and patients who have diabetes in whom hypoglycemia
can occur very rapidly and be detrimental.
III. Hemodilution
One common accompaniment of fluid administration is
a reduction in the hemoglobin and hematocrit. With
active blood loss, the use of asanguineous fluids
can cause marked anemia. An increase in cerebral
blood flow (CBF) typically accompanies this
hemodilution, and physicians have long argued
whether the hemodilution is beneficial, benign, or
detrimental. The answer probably depends on the
degree of hemodilution and on the state of the
disease.
From a theoretical vantage, a hematocrit of 30% to
33% gives the optimal combination of viscosity and
oxygen-carrying capacity. In the normal brain, the
increase in CBF produced by hemodilution is almost
certainly an active compensatory response to a
decrease in arterial oxygen content; this response
is essentially identical to that seen with hypoxia.
With a brain injury, however, the normal CBF
response to hypoxia and hemodilution is attenuated,
and both conditions can contribute to secondary
tissue damage.
The one situation in which hemodilution might be
beneficial is the period during and immediately
after a focal cerebral ischemic event. Several
animal studies have shown that regional oxygen
delivery may be increased (or at least better
maintained) in the face of modest hemodilution to a
hematocrit of approximately 30% with improvement in
CBF and reduction in infarction volume. In spite of
this, several clinical trials have failed to
demonstrate any benefit from hemodilution in stroke
patients, except in those who were polycythemic to
begin with. The lack of success, however, may
reflect the delay in instituting therapy or
inadequate hematocrit reduction.
What clinical lesson can be learned from the work on
hemodilution? Our opinion is that for elective
neurosurgical patients and patients suffering from
head injuries, hemodilution to a hematocrit below
30% to 35% is unlikely to be any more “beneficial”
than hypoxia. Hemodilution to 30% to 35% might be
better tolerated in patients who are at risk for
focal ischemia. Nevertheless, active attempts to
reduce the hematocrit below 30% are probably not
advisable at the present time.
Water and electrolyte disturbances. Table-3
summarizes the principal differences among the most
common water and electrolyte disturbances in
patients who have intracranial pathology.
Diabetes insipidus (DI). DI is a common sequela of
pituitary and hypothalamic lesions, but it can also
occur with other cerebral pathology, such as head
trauma, bacterial meningitis, intracranial surgery,
phenytoin use, and alcohol intoxication. Patients
who have markedly elevated ICP and brain death also
commonly develop DI.
Table-3. Principal water-electrolyte disorders |
Factor |
DI |
SIADH |
CSW
|
Etiology |
Reduced secretion
of ADH |
Excessive release
of ADH |
Release of brain natriuretic factor |
Urine |
Output |
>30 mL/kg/hr |
|
|
Specific gravity |
<1.002 |
|
|
Sodium |
<15 mEq/L |
>20 mEq/L |
>50 mEq/L |
Osmolality vs.
serum osmolality |
Lower |
Higher |
Higher |
Serum |
Sodium |
Hypernatremia |
Hyponatremia |
Hyponatremia |
Osmolality |
Hyperosmolality |
Hypoosmolality |
Intravascular
volume |
Reduced |
Normal or increased |
Reduced |
DI, diabetes insipidus; SIADH, syndrome of
inappropriate antidiuretic hormone secretion; CSW,
cerebral salt wasting syndrome; ADH, antidiuretic
hormone. |
DI is a metabolic disorder caused by the decreased
secretion of antidiuretic hormone (ADH). This
results in the failure of the tubular reabsorption
of water. Polyuria (>30 mL/kg/hour or, in an adult,
>200 mL/hour), progressive dehydration, and
hypernatremia occur subsequently. DI is present when
the urine output is excessive, the urine osmolality
is inappropriately low relative to serum osmolality
(which is above normal because of water loss), and
the urine specific gravity is <1.002.
Management. The management of DI requires
restoration of normal serum sodium and carefully
balancing intake and output to avoid fluid overload.
The patient should receive hourly maintenance fluids
plus either three-fourths of the previous hour's
urine output or the previous hour's urine output
minus 50 mL (Table-4). Half-normal saline and
free water are commonly used as replacement fluids,
with appropriate potassium supplementation. Serum
sodium, potassium, and glucose should be checked
frequently.
If the urine output is >300 mL/hour for 2 hours, it
is now standard practice to administer aqueous
vasopressin, 5 to 10 international units of drug
(IU), intramuscularly (i.m.) or subcutaneously (s.c.)
every 6 hours or the synthetic analog of ADH,
desmopressin acetate, 0.5 to 2 mcg intravenously
(i.v.) every 8 hours or by nasal inhalation, 10 to
20 mcg.
Syndrome of inappropriate antidiuretic hormone
secretion (SIADH). Various cerebral pathologic
processes (mostly head trauma) can cause excessive
release of ADH, which leads to the continued renal
excretion of sodium (>20 mEq/L), despite
hyponatremia and associated hypo osmolality. Urine
osmolality is therefore high relative to serum
osmolality. SIADH can also result from the excessive
administration of free water in patients who cannot
excrete free water because of excess ADH.
Management. The mainstay of treatment of SIADH is
fluid restriction to 1,000 mL/24 hours of iso-osmolar
solution. If hyponatremia is severe (<110 to 115
mEq/L), the administration of hypertonic (3% to 5%)
saline and furosemide might be appropriate. Because
rapid correction of hyponatremia has been associated
with the occurrence of central pontine myelinolysis,
restoring serum sodium at a rate of approximately 2
mEq/L/hour is advisable.
Table -4. Management of diabetes insipidus |
Hourly monitoring of UO
Maintenance fluids + 75% of the previous hour's UO
or
Maintenance fluids + the previous hour's UO - 50 mL.
If UO >300 mL/hr: vasopressin or desmopressin
|
UO, urinary output.
|
Cerebral salt wasting syndrome (CSW). CSW is
characterized by hyponatremia, volume contraction,
and high urine sodium concentration (>50 mEq/L).
This syndrome is frequently seen in patients after
subarachnoid hemorrhage (SAH). The causative factor
seems to be the increased release of a natriuretic
factor from the brain.
Management. The therapy is to reestablish
normovolemia with the administration of
sodium-containing solutions.
The distinction between SIADH and CSW is very
important because treatment of these two syndromes
is quite different: fluid restriction versus fluid
infusion, respectively. It should be stressed that
in patients who have SAH for whom normo- to
hypervolemia is advocated, fluid restriction (i.e.,
further volume contraction) might be especially
deleterious.
IV. Conclusion
As neuroanesthesiologists and
intensivists, we should always remember that we
treat the whole patient, not only the brain.
Therefore, with the exception of patients with
SIADH, we should abandon the old dogma that patients
who have intracranial pathology must be "run dry"
and replace it with "run them isovolemic, isotonic, and iso-oncotic."
V. Key points
Movement of water between the normal brain and the
intravascular space depends on osmotic gradients.
Reducing serum osmolality by administering free
water or hypotonic crystalloid solutions (0.45%
NaCl) results in edema formation in all tissues,
including normal brain tissue.
Reduction of COP with maintenance of serum
osmolality is associated with increased water
content in many tissues, but not in the normal
brain. Colloid solutions exert little influence on
brain water content and ICP.
In the setting of brain injury, reducing serum
osmolality increases edema and ICP. Therefore, the
goal of fluid management in neurosurgery is to avoid
the reduction of serum osmolality. Reduction of COP,
with careful maintenance of osmolality, does not
increase edema in the injured brain.
Hypertonic solutions: mannitol decreases brain water
content in the normal brain and is commonly used to
reduce ICP. HS decreases brain water content and ICP
but can cause hypernatremia.
Glucose-containing solutions should not be used in
patients who have brain pathology and should be
avoided in patients at risk for cerebral ischemia.
Fluid restriction minimally affects cerebral edema
and, if overzealously pursued, can lead to
hemodynamic instability, which is detrimental in
neurosurgical patients.
Isotonic crystalloid solutions are widely used to
maintain and/or restore intravascular volume.
|